
Piezoelectric Dynamic Therapy (PzDT) is a tumor treatment method that utilizes mechanical energy to trigger the polarization of piezoelectric materials. Under the action of ultrasound (US), the immense pressure generated by the collapse of cavitation bubbles serves as a mechanical force source for the piezoelectric sonosensitizer, showing feasible potential. An internal electric field can form within the piezoelectric material, leading to band bending, electron-hole pair separation, and redox reactions in the tumor microenvironment. The carrier concentration, carrier recombination rate, and active sites of the piezoelectric material can affect the piezoelectric performance of the material, thereby influencing the efficiency of PzDT. Various methods can be employed to enhance the efficiency of PzDT, such as using doping engineering to modify the crystal structure, phase composition, and grain size. Doping engineering not only affects the piezoelectric performance of the material, but some dopant ions can react with the overexpressed H2O2 in the tumor microenvironment to generate toxic ·OH, significantly increasing the yield of reactive oxygen species (ROS). Oxygen vacancy engineering plays an extremely important role in enhancing the catalytic performance of nanomaterials. Oxygen vacancies can significantly alter the electronic structure of semiconductors, improving charge transport capabilities, especially in two-dimensional nanomaterials, where oxygen vacancies can greatly enhance catalytic performance. Oxygen vacancies can be classified into surface oxygen vacancies and bulk oxygen vacancies. Surface oxygen vacancies can serve as adsorption and activation sites for reactants on the material’s surface, enhancing the catalytic activity and reaction rate of the material. Therefore, the method of creating surface oxygen vacancies to enhance the reaction rate of chemical dynamic therapy (CDT) is highly attractive. Oxygen vacancies can introduce new localized states at the conduction band or valence band edge, effectively modulating the band structure and suppressing surface charge recombination, facilitating easier separation of electrons and holes. Consequently, fabricating piezoelectric sonosensitizers with oxygen vacancies can optimize tumor treatment outcomes.
BiOCl (BOC) is a special ternary oxide widely used due to its low toxicity, good chemical stability, and easy tunability of electronic structure. Its unique anisotropic layered structure aids in forming two-dimensional nanosheets with highly exposed surfaces. Most atoms in two-dimensional materials are located at the surface, making it easier to adsorb atoms or construct defects on the material’s surface, thus accelerating reactions. Additionally, BOC exhibits significant piezoelectric properties, generating charge separation under external forces due to the non-coincidence of its internal positive and negative charge centers, thereby enabling the conversion of mechanical energy into electrical energy.
Recently, Professor Yang Piaoping’s team from Harbin Engineering University designed two-dimensional Cu-doped BiOCl nanosheets with surface oxygen vacancies (SV-CBOC NSs) for synergistic cancer treatment (Figure 1). They constructed surface oxygen vacancies on these perovskite-structured nanosheets using a photolithography strategy, serving as electron traps that effectively suppress electron-hole recombination. By modulating the polarization state within the nanosheets using the pressure generated by US, an internal electric field is formed, thereby enhancing the separation efficiency of electrons and holes. Electrons can reduce O2 to generate O2·−, leading to the death of tumor cells. Meanwhile, Cu doping and the transfer of electrons to Cu2+ can enhance the decomposition of H2O2, thereby increasing ·OH generation and producing more ROS. This perfect combination of PzDT, SDT, and CDT intensifies the lethality against tumor cells, providing new directions and references for US-mediated multimodal tumor therapy.
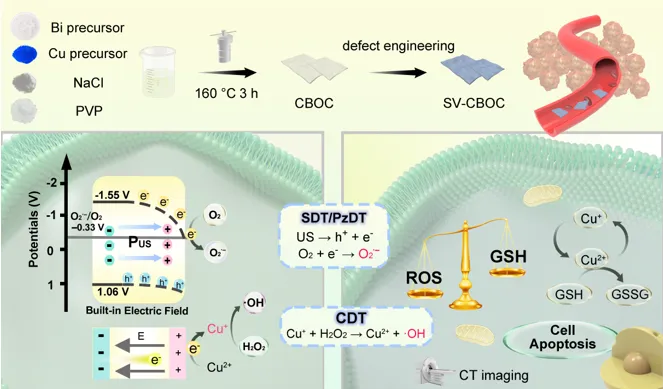
Figure 1 Schematic diagram of the preparation of SV-CBOC NSs and their synergistic therapeutic mechanism.
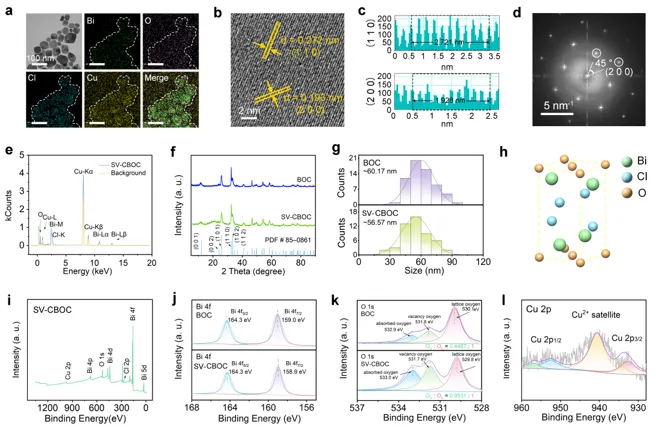
Figure 2 Characterization of the prepared BOC and SV-CBOC NSs. a) TEM and element mapping of SV-CBOC NSs. b, c) High-resolution TEM images and interplanar spacing of SV-CBOC NSs. d) SAED pattern corresponding to SV-CBOC NSs. e) EDS spectrum and background baseline of SV-CBOC NSs. f) XRD spectrum. g) Particle size distribution of BOC and SV-CBOC NSs. h) Crystal structure of BOC. i) XPS measurement spectrum of SV-CBOC. j-1) High-resolution XPS spectra of Bi 4f, O 1s, and Cu 2p for SV-CBOC.
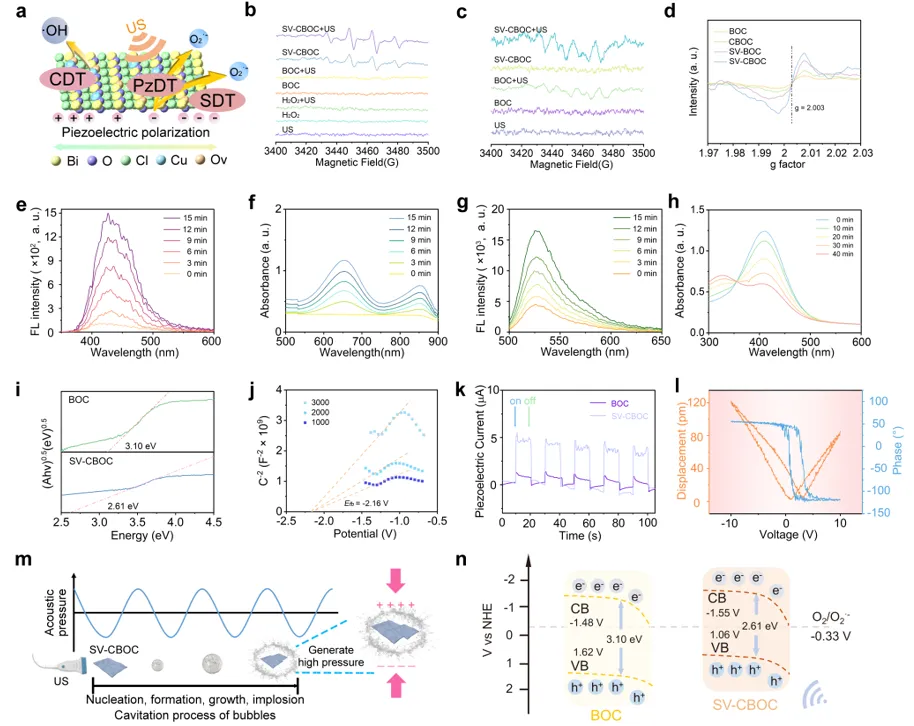
Figure 3 a) Schematic diagram of CDT, PzDT, and SDT for SV-CBOC NSs. b, c) ESR spectra of ·OH and O2·− captured by DMPO under different conditions. d) ESR spectra showing the defect structures of BOC, CBOC, SV-BOC, and SV-CBOC NSs. e) Fluorescence spectrum detecting the ability of SV-CBOC NSs to generate ·OH under US. f) Time-dependent absorbance of TMB for SV-CBOC NSs under US irradiation. g) Fluorescence spectrum of DHR123 detecting the generation of O2·− by SV-CBOC NSs under US. h) Time-dependent absorbance spectrum of DTNB after treatment of SV-CBOC NSs under US. i) Band gap of BOC and SV-CBOC NSs. j) Mott-Schottky curve of SV-CBOC NSs. k) Piezoelectric current curves of BOC and SV-CBOC NSs. l) PFM characterization of SV-CBOC NSs (amplitude butterfly loop and phase hysteresis loop). m) Schematic diagram of the piezoelectric effect of SV-CBOC NSs under US stimulation. n) Theoretical schematic diagram of the energy band of BOC and SV-CBOC NSs.
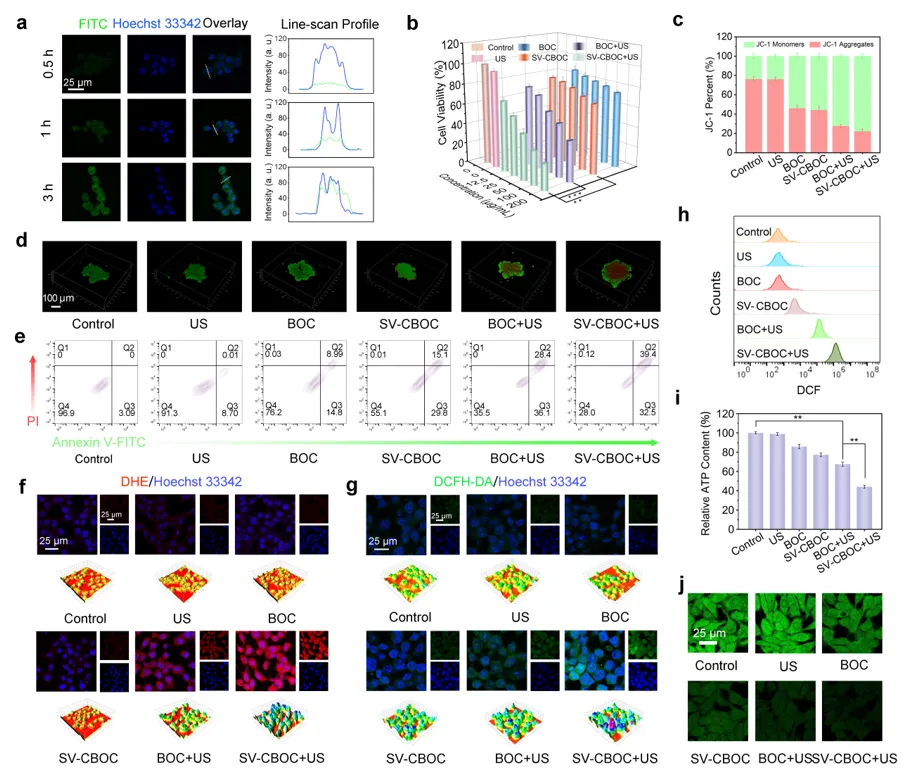
Figure 4 a) Cellular uptake of FITC-labeled SV-CBOC NSs by 4T1 cancer cells after different incubation time intervals. b) Cell viability of 4T1 cancer cells under different treatments. c) Fluorescence correlation of monomers and aggregates in JC-1 probe. d) Confocal images of 4T1 cancer cells stained with Calcein-AM and PI after different treatments. e) Flow cytometry apoptosis analysis of Annexin V-FITC/PI stained cells under different treatments. f, g) Levels of O2·− and ROS in cells under different treatments. h) Flow cytometry analysis of intracellular ROS generation in 4T1 cells after different treatments. i) Relative ATP levels of 4T1 cancer cells measured by ATP assay kit after different treatments. j) Depletion of GSH in 4T1 cells under different treatments.
Figure 5 a) Detailed flowchart of SV-CBOC NSs imaging. b) In vitro CT pseudo-color images of SV-CBOC NSs dissolved in PBS at different concentrations. c) In vitro CT values of SV-CBOC NSs at different concentrations. d) CT images of tumor-bearing mice before and after injection of SV-CBOC solution and corresponding CT values along tumor slices. e) Cross-sectional CT images of tumor-bearing mice after intravenous injection of SV-CBOC NSs at different time intervals. f) Time-dependent CT values of the tumor area after intravenous injection of SV-CBOC NSs. g) Schematic diagram of the anti-tumor effect of SV-CBOC NSs. h) Bi distribution in major tissues and tumors at 3, 6, 12, 24, 48 h after intravenous injection of SV-CBOC solution. i) Blood circulation curve after intravenous injection of SV-CBOC solution. j) Blood circulation elimination rate curve of SV-CBOC. k) Individual tumor growth curves of tumor-bearing mice in different treatment groups (n = 5). l) Body weight of mice in each treatment group (n = 5). m) Corresponding photos of excised tumors. n) H&E and TUNEL stained images of tumor tissues in different treatment groups.
Professor Yang Piaoping’s team designed ultra-thin CBOC NSs with numerous surface oxygen vacancies through doping engineering and defect engineering strategies. US stimulation triggers piezoelectric responses, promoting ROS generation while accelerating the conversion of Cu (II) to Cu (I) and the oxidation of GSH. The surface oxygen vacancies generated after photolithography significantly enhance catalytic efficiency, reduce band gap, and improve carrier separation rates. Through Cu doping, ROS generation is increased, and H2O2 in the tumor microenvironment is reduced, further enhancing the therapeutic effect. This research not only converts US signals into electrical signals but also combines with other therapeutic modalities to achieve effective anti-tumor effects, enhancing the application prospects of piezoelectric materials in the field of tumor therapy through doping engineering and defect engineering.
Related work titled “Oxygen vacancy piezoelectric nanosheets constructed by photo-etching strategy for ultrasound ‘unlocked’ tumor synergistic therapy” was published in Nano Letters. Professor Yang Piaoping from Harbin Engineering University, Associate Professor Feng Lili, and Associate Chief Physician Xu Rongchen from the PLA General Hospital are the corresponding authors of this paper.
Paper link:
https://pubs.acs.org/doi/10.1021/acs.nanolett.4c01656
(Click the lower left corner “Read the original text” to go directly to the original text)
For submissions, reprints, and collaborations, please contact WeChat:MatResFron001
Welcome to leave a message and share your views.Support the author by clicking the lower right corner “Like”” and “Looking”↓↓↓