Research progress of polyimide as electrode materials for supercapacitors
DING Chengcheng ,YU Juan ,HUANG Pei ,WANG Xiaodong
(State Key Laboratory of Materials-Oriented Chemical Engineering,College of Chemical Engineering,Nanjing Tech University,Nanjing 211800,China)
DOI:10.3969/j.issn.1001-3539.2024.01.025
Fund Information: National Natural Science Foundation Project (22035007)



1.1 Transition Metal Oxides
1.2 Conductive Polymers
1.3 Carbon Materials
2 Polyimide-based Electrodes
2.1 PI Porous Carbon Electrode Materials
3 Summary and Outlook
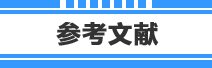
1 TAN J,et al. Energy & Environmental Materials,2020,4:302‒306.
2 YU L P,et al. Electrochemical Energy Reviews,2020,3:271‒285.
3 Wang Huiying,et al. Modern Chemical Industry,2023,43(3):30‒35.
4 LIU S,et al. Applied Energy,2020,278. DOI:10.1016/j.apenergy.2020.115436.
5 Wang Wenhao,et al. Guangzhou Chemistry,2022,47(6):1‒10.
6 CHERUSSERI J,et al. Nanotechnology,2019,30. DOI:10.1088/1361-6528/ab0685.
7 POONAM,et al. Journal of Energy Storage,2019,21:801‒825.
8 ZHAO Z,et al. Carbon,2023,210. DOI:10.1016/j.carbon.2023.118066.
9 DELBART S A,et al. Journal of Alloys and Compounds,2021,857. DOI:10.1016/j.jallcom.2020.158281.
10 MA Y,Hou C,Kimura H,et al. Advanced Composites and Hybrid Materials,2023,6. DOI:10.1007/s42114-023-00636-1.
11 Wang Kangyu,et al. New chemical materials,2023,51(Suppl.1):1‒6.
12 MOHD ABDAH M A A,et al. Materials & Design,2020,186. DOI:10.1016/j.matdes.2019.108199.
13 Cai Jiangtao,et al. Engineering Plastics Application,2022,50(2):7‒13.
14 WANG C,et al. Applied Surface Science,2023,612. DOI:10.1016/j.apsusc.2022.155661.
15 SHEN B,et al. Applied Surface Science,2023,609. DOI:10.1016/j.apsusc.2022.155189.
16 WANG Y,et al. Nano Research,2019,12(9):1 978‒1 987.
17 ZHAO C,et al. Journal of Materials Chemistry A,2020,8(9): 4 677‒4 699.
18 宋慧敏,等. Engineering Plastics Application,2022,50(2):21‒26.
19 SUN L,et al. Journal of Energy Storage,2023,61. DOI:10.1016/j.est.2023.106738.
20 ZHAI Z,et al. Materials & Design,2022,221. DOI:10.1016/j.matdes.2022.111017.
21 梁昱巍,等. 材料工程,2023,51(6):38‒45.
22 KUMAR S,et al. Chemical Engineering Journal,2021,403. DOI:10.1016/j.cej.2020.126352.
23 俞娟,等. 南京工业大学学报(自然科学版),2019,41(4):403‒410.
24 LYU C,et al. Energy,2023,270. DOI:10.1016/j.energy.2023.126830.
25 WU Q,et al. Applied Surface Science,2017,425:1 082‒1 088.
26 LI Z,et al. Journal of Energy Storage,2021,44. DOI:10.1016/j.est.2021.103437.
27 IN J B,et al. Carbon,2015,83:144‒151.
28 QING Y,et al. Journal of Materials Chemistry A,2019,7(11): 6 021‒6 027.
29 RUAN X J,et al. Materials and Design,2018,160:1 168‒1 177.
30 WANG S,et al. Electrochimica Acta,2017,241:153‒161.
31 KIM H,et al. Energies,2021,14. DOI:10.3390/en14092547.
32 LIU Y,et al. Int J Energy Res.,2022,46:370‒382.
33 LIU Y,et al. ACS Appl. Energy Mater.,2022,5:1 205‒1 217.
34 YU J,et al. Materials Letters,2023,339. DOI:10.1016/j.matlet.2023.134036.
35 LI H,et al. Journal of Colloid and Interface Science,2022,609:179‒187.
36 WU M,et al. Chemical Engineering Journal,2023,466. DOI:10.1016/j.cej.2023.143077.
37 XU Z,et al. Advanced Materials,2016,28(10):1 981‒1 987.
38 LEE S,et al. Advanced Sustainable Systems,2018,2. DOI:10.1002/adsu.201800007.
39 PENG H,et al. Journal of Energy Storage,2020,30. DOI:10.1016/j.est.2020.101493.
40 PENG H,et al. Journal of Power Sources,2021,482. DOI:10.1016/j.jpowsour.2020.228993.
41 WANG Y,et al. Journal of Energy Storage,2022,55. DOI:10.1016/j.est.2022.105390.
42 WU S,et al. Journal of Energy Storage,2023,71. DOI:10.1016/j.est.2023.108152.
43 LIU X,et al. Electrochimica Acta,2023,454. DOI:10.1016/j.electacta.2023.142417.
44 LIU X,et al. Energy Technol. 2020,8. DOI:10.1002/ente.201901013.
45 WANG Y,et al. Journal of Materials Science,2020,56(1):173‒200.
46 ZHANG Y,et al. RSC Adv.,2015,5. DOI:10.1039/C4RA13015D.
47 ZHANG Y,et al. Advanced Materials Interfaces,2016,3. DOI:10.1002/admi.201600137.
48 WANG D,et al. Electrochimica Acta,2019,323. DOI:10.1016/j.electacta.2019.134811.
49 ZHANG Y,et al. Electrochimica Acta,2018,283:1 763‒1 772.
50 LAI F,et al. Journal of Materials Chemistry A,2016,4:15 861‒15 869.
51 ZHAO X,et al. Nanotechnology,2020,31. DOI:10.1088/1361-6528/ab7585.
52 ZHENG Z,et al. Gels,2022,8. DOI:10.3390/gels8050308.
53 DING C,et al. Journal of Materials Science,2022,57(20):9 480‒9 492.
54 DING C,et al. Journal of Materials Science,2022,57(47):21 680‒21 692.
55 夏恒恒,等. 电子元件与材料,2022,41(12):1 272‒1 285.
56 Wang Yao,et al. Journal of Materials Engineering,2021,49(9):51‒59.
57 KIM D K,et al. Journal of Power Sources,2018,380:55‒63.
58 PARK G-T,et al. Materials Science and Engineering B,2022,275. DOI:10.1016/j.mseb.2021.115530.
59 XU H,et al. RSC Advances,2022,12:21904–21915.
60 CHEN L,et al. ACS Applied Energy Materials,2022,5:1580–1594.
61 LIU S,et al. RSC Advances,2018,8:25568–25574.
62 LI R,et al. Journal of Power Sources,2023,573. DOI:10.1016/j.jpowsour.2023.233113.
63 YAN B,et al. Diamond & Related Materials,2022,130. DOI:10.1016/j.diamond.2022.109465.
64 CHO Y H,et al. Molecules,2020,25. DOI:10.3390/molecules25245863.
65 HAN N K,et al. Journal of Applied Polymer Science,2019,136. DOI:10.1002/app.47846.
66 HAN N K,et al. Composites Science and Technology,2020,196. DOI:10.1016/j.compscitech.2020.108212.
67 WANG Z,et al. Polymer,2022,253. DOI:10.1016/j.polymer.2022.124945.
68 LEE D G,et al. Polymers,2021,13.DOI:10.3390/polym13050720.
69 XU W,et al. Journal of Energy Storage,2022,47. DOI:10.1016/j.est.2021.103672.
70 LE T,et al. Carbon,2018,138:325‒336.
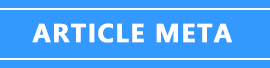