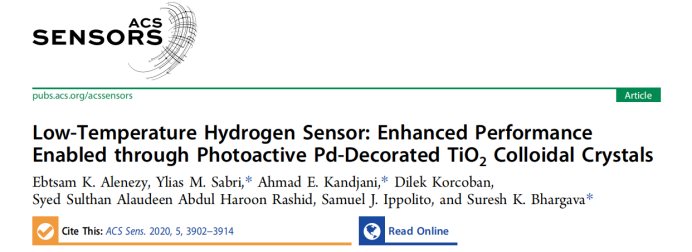
Hello everyone, today I would like to share a paper published in 2020 in ACS Sensors, titled “Low-Temperature Hydrogen Sensor: Enhanced Performance Enabled through Photoactive Pd-Decorated TiO2 Colloidal Crystals”. This research indicates that using a sensitive layer based on Pd modified TiO2 long-range ordered crystals in a photo-assisted amperometric gas sensor can achieve excellent H2 sensing performance. The unique combination of this material and its novel layered structure allows for the detection of H2 gas at 50 ppm, with a promising LoD capability. The sensor response profile shows that under a 9V bias (relative to other conditions used), the sensor has a higher signal-to-noise ratio in the presence of light, producing a LoD of only 3.5 ppm at an operating temperature of 33℃. The high performance of this sensor makes it attractive for applications that require low-level (ppm compared to conventional % levels) H2 gas detection. Importantly, the developed sensor shows high selectivity to carbon dioxide, methyl ethyl ketone, acetone, acetaldehyde, and nitric oxide (>93%). The first author of this paper is Ebtsam K. Alenezy, and the corresponding author is Ylias M. Sabri.
The high demand for H2 gas sensors is not limited to industrial process control and leak detection applications, but also extends to the food and medical industries to determine the presence of various types of bacteria or potential medical conditions. For example, sensing low concentrations (<10 ppm) of H2 is crucial for developing non-invasive diagnostic breath analyzers for some gastrointestinal diseases. However, achieving high sensitivity for H2, thereby reducing the detection limit (LoD), still presents significant challenges that need to be overcome.
Palladium decoration on titanium dioxideLROCs plays an important role in the catalytic conversion of H2 gas. When Pd modified titanium dioxideLROCs are used as the sensitive layer for photo-assisted amperometric H2 gas sensing, they exhibit impressive sensing performance. In contrast, the original titanium dioxide thin films, titanium dioxideLROCs, and control sensors based on Pd seeded titanium dioxideLROC (low Pd content) do not generate readable sensor output when exposed to H2 gas under any of the above conditions. Pd NPs also provide a pathway that can reduce activation energy, thereby improving reaction rates, sensitivity, selectivity, and reliability. Pd NPs can also act as H2 adsorbents, delivering H2 to the TiO2/Pd interface, facilitating the reaction, which can be explained by the spillover effect described elsewhere. When oxygen (in dry air) interacts with the surface of the developed Pd modified titanium dioxideLROC, oxygen captures electrons from the conduction band surface of the sensitive layer, forming ionic species such as O−, O2−, and O2−. This leads to an increase in the sensor’s resistance as the depletion layer increases. Well-dispersed Pd NPs promote an increase in gas response by creating active sites (electron sensitization), as the Schottky barrier between Pd NPs and titanium dioxideLROCs serves as a better dissociation catalyst than using titanium dioxide alone. Similarly, a Schottky junction also occurs between the n type titanium dioxideLROCs and the platinumIDT sensor electrode. The resulting Schottky barrier height prevents electrons from flowing from titanium dioxideLROCs to the metal (Pd NPs and Pt electrode) without the aid of external energy, raising their energy above the Schottky barrier height. Thus, a bias voltage is required to be reached for operation. Under the influence of different biases, the materials exhibit different adsorption equilibria on the surface. When a bias is applied, the semiconductor band will flow electrons into the metal, enhancing the sensor’s performance and increasing the response amplitude. Furthermore, as the bias increases from 0.1 to 9V, the response amplitude increases, which may be attributed to AGS being in a diffusion-controlled mode rather than an electrode kinetics-controlled mode.
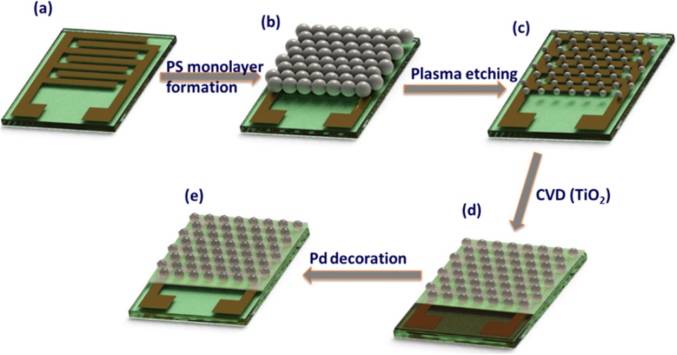
Figure 1 Preparation Process of Pd-TiO2 shows a schematic diagram of the manufacturing process of using Pd decorated titanium dioxideLROCs on the sensor substrate. In simple terms, the sensor substrate (Figure 1a) is covered with PS LROCs, forming a uniform mask through colloidal photolithography (Figure 1b). It is then uniformly etched with a plasma cleaner to modify the particle size of PS LROCs (Figure 1c). The next step is to deposit a uniform titanium dioxide thin film on the LROC-covered substrate using CVD. The substrate is then annealed at 550℃ to remove the polystyrene template. The annealing process simultaneously crystallizes the amorphous titanium dioxide material deposited on the LROCs (Figure 1d). Finally, through the ELP process, uniform Pd NPs decorate the TiO2 LROCs (Figure 1e).
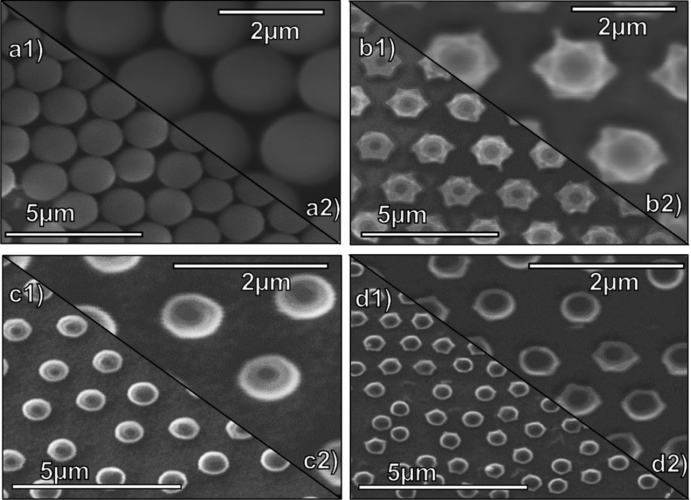
Figure 2 Morphological Characterization of the Device
Low-magnification and high-magnification electron microscope images of hexagonally closed and non-closed PS monolayers are shown in Figures 2a and b, respectively. Scanning electron microscope images obtained at the same magnification analyzed the particle size distribution of the PS template before and after etching. The images of non-closely packed TiO2/PS LROCs after 10 min of CVD and after annealing in air at 550℃ for 1 h are shown in Figures 2c and d, respectively. It can be observed that there is no significant change in the structure and distance between the colloids during the annealing process. When polystyrene degrades above 418.6℃, hollow titanium dioxide microshell structures are formed in the annealed LROCs.
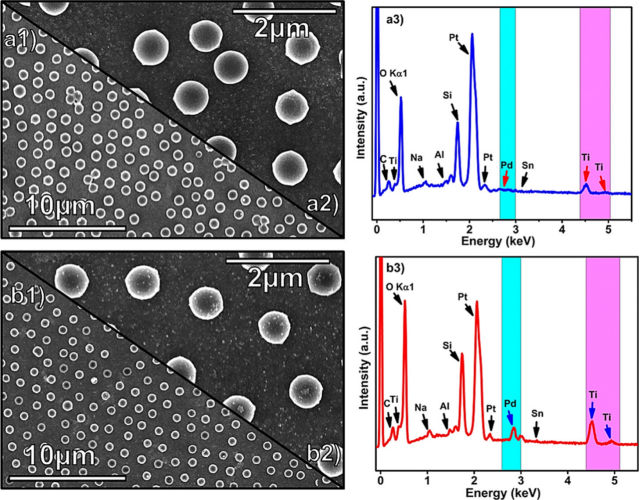
Figure 3 Scanning Electron Microscopy Images of Pd NPs Seeded and Decorated on Titanium Dioxide
Figure 3 (a1 and a2) shows that the Pd seeded titanium dioxide LROCs do not display any visible Pd NPs due to the small particle size. The Pd modified titanium dioxide LROCs in Figure 3b shows uniform Pd particles deposited on the surface of the titanium dioxide LROC. The results indicate that the Pd particles in the ELP process have more Pd NPs than the seeded samples, achieving higher H2 permeability and higher thermal stability. To further confirm the formation of Pd NPs and investigate the impurity content introduced during the synthesis process, all samples were studied using energy dispersive X-ray spectroscopy (EDS). EDS results indicate the presence of titanium, oxygen, and palladium in the samples, confirming the successful deposition of Pd on titanium dioxide LROCs with no impurities present.
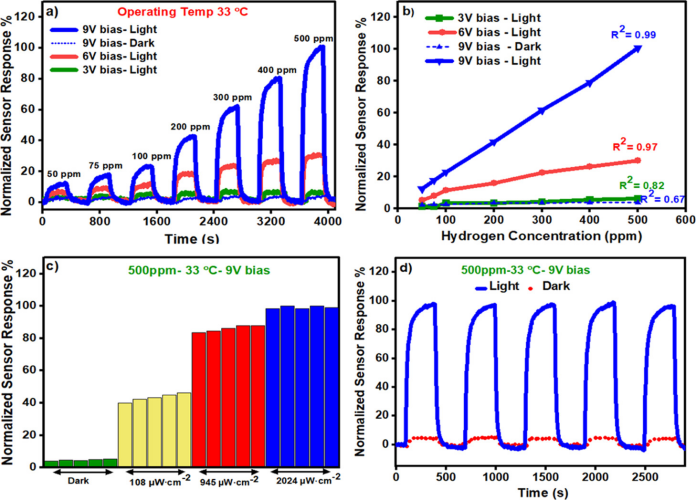
Figure 4 Device Performance Testing
To explore the possibility of the developed materials as high selectivity and sensitivity materials for H2 gas sensing, the researchers used them as sensitive layers on AGSs. Additionally, photo-assisted H2 sensing tests were also conducted to achieve low-temperature gas sensing. The developed sensor’s sensing performance was tested by exposing it to 50, 75, 100, 200, 300, 400, and 500 ppm of H2 gas concentration. Using a computer-controlled gas delivery device, the sensing performance of titanium dioxide thin films (control sensors) and titanium dioxideLROCs was tested. The normalized response of the Pd modified sensor is shown in Figure 4a. The calibration curves of the developed pd modified titanium dioxide LROC sensor at different bias voltages are shown in Figure 4b. The data indicates that the sensor’s response under illumination is higher than in dark conditions, increasing from 50 ppm to 500 ppm, from ∼55 to ∼91%. Lowering the bias reduces the sensor’s usability under dark conditions (9 < bias ≤3 V), and the sensor’s response to H2 increases with the applied bias. Moreover, under a bias of 0.1 V, the sensor does not produce any response to H2 (under either light or dark conditions). The data shows that at the highest applied voltage of 9V, the photo-assisted H2 sensing achieved the highest sensor response amplitude. Under illumination at 365 nm, 2024μW·cm−2, the response amplitude is linear (R2 = 0.99), with a calculated sensitivity of S=6.2μA/ppm) (see Figure 4b). The developed sensor, by exposing to 500 ppm of H2, under different continuous pulses, with a dark intensity at a 9V bias, at a working temperature of 33℃ (Figure 4c). The data indicates that the increase in light intensity leads to the generation of relatively more electron-hole pairs, resulting in the formation of light-induced oxygen ions, thus increasing the response amplitude to H2 gas. The results in Figure 4d show that the developed Pd decorated titanium dioxide LROC sensor exhibited good repeatability of 99.9% in the absence of ultraviolet illumination at 9V.
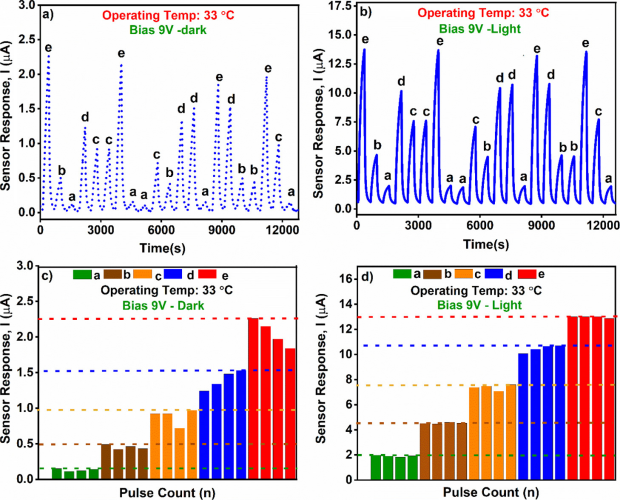
Figure 5 Memory Effect of the Device
To determine whether the developed sensor is affected by previous sensing events, five different hydrogen concentrations (called a, b, c, d, and e), i.e., = 100, b = 200, c = 300, d = 400, and e = 500 ppm were used. Each concentration was repeated at least 4 times, while the other four test concentrations were repeated at least once. Figures 5a and b show the sensor’s response curves during memory tests at 33°C, while the analytical graphs (response amplitude) of the same test are shown in Figures 5c and d. Interestingly, it was found that under ultraviolet light, the memory effect is significant. However, as shown in Figure 5d (bar chart representation), a slight memory effect is observed even in dark conditions (<1%). It is clear that regardless of the sequence of exposure, the sensor demonstrates similar response amplitudes for each repeated H2 gas concentration detected. This remains true even when testing the lowest H2 gas concentration (100 ppm) after testing the highest H2 gas concentration (500 ppm).
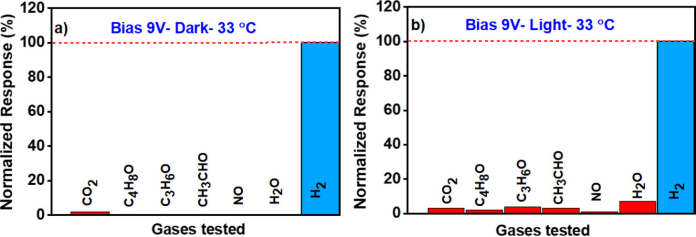
Figure 6 Selectivity Data of the Sensor under Dark Conditions and UV Illumination
The selectivity performance of the sensor was tested by exposing the Pd modified titanium dioxideLROC sensor to common H2 and various concentrations of interfering gases in most industries. These tests were conducted in the presence of air, at an operating temperature of 33℃, both in the presence and absence of light. The types of gases included carbon dioxide (500 ppm), methyl ethyl ketone (26.1 ppm), acetone (25 ppm), acetaldehyde (3034 ppm), and NO (2000 ppm), most of which were tested at the expected maximum concentration in industrial processes. Figures 6a and b show the response of the sensor when exposed to each gas in air. The results indicate that the response of the developed sensor to H2 is higher than to other gas tests, both in the absence and presence of light due to selective adsorption of H2 by Pd NPs. The developed sensor showed no significant sensitivity to other gases under repeated conditions, due to the specific selectivity of Pd NPs for H2 gas. Additionally, it was observed that both selectivity and sensitivity can be controlled by the presence/absence of light, indicating that the developed sensor can be used for a wide range of hydrogen concentrations (from low ppm to high % levels). The lowest selectivity experienced by the sensor was due to humidity exposure, resulting in an acceptable selectivity of 93% under the presence of ultraviolet illumination. However, under dark conditions, a readable response amplitude was observed for carbon dioxide, but still with a selectivity output of 98% for H2 gas. When the metal oxide (titanium dioxideLROCs) sensor operates in a humid atmosphere, the adsorption of water vapor and molecular oxygen releases electrons to the depletion layer, leading to a decrease in resistance and an increase in surface current of the oxide, which may change the sensor’s baseline current when dry air becomes humid air.
With Pd NPs decorated directly deposited on AGS devices, the titanium dioxide LROC sensitive layer was developed. To improve the sensor’s sensitivity and selectivity to H2 gas near room temperature, the sensor was studied under different ultraviolet light and bias conditions. The developed Pd decorated titanium dioxide LROC sensor’s LoD decreases with increasing applied bias. Under illumination, the LoD at a 9V bias is 3.5 ppm, much lower compared to 6 and 3V bias conditions. This is due to the use of Pd NPs as dissociation catalysts and the application of external stimuli (i.e., bias and illumination), leading to an increase in the reaction rate and electron flow, thereby increasing the H2 reaction rate on the catalytic sensitive layer. The data indicates that this method allows for high selectivity detection of low hydrogen concentrations (50 ppm) at close to RT (33°C). The developed sensor’s ability to detect low ppm levels makes it suitable for various applications, including breath analysis (non-invasive diagnostics), industrial leak testing, and process control.
1. Low temperature high sensitivity: Based on palladium-decorated TiO₂ colloidal crystals, the sensor also exhibits high hydrogen sensitivity at low temperatures, benefiting from the catalytic and photoactive properties of palladium and TiO₂.
2. Photocatalytic effect: Ultraviolet illumination enhances sensor performance, with the photocatalytic action of TiO₂ accelerating reaction rates and improving detection efficiency.
3. Optimized structure ensures stability: The colloidal crystal structure enhances the stability and repeatability of the sensor, making it a strong candidate for hydrogen detection.
Original Link
https://dx.doi.org/10.1021/acssensors.0c01387
For submissions, recommendations, and collaborations, please contact us!
|| WeChat Official Account: Micro-Nano Sensors
|| WeChat ID: successplus999
Micro-Nano Sensor Industry Research
Academic Frontier Research Reports
Author: Tang Kaolan
Reviewer: Teacher Li
Promoter: Tian Tiantian
Statement ————
This article is for research sharing only and is not for profit. If there is any infringement, please contact the backend staff for deletion.
Due to limited knowledge, there may be omissions and errors. Please feel free to criticize and correct.