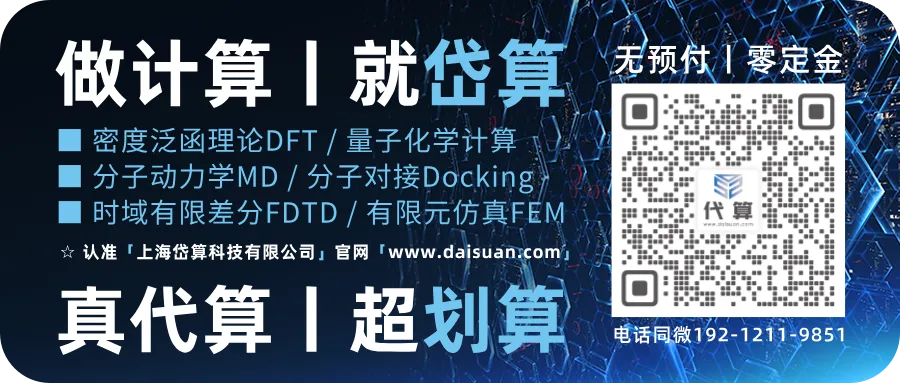
Reasonable adjustment of the electronic structure of sulfur electrocatalysts is crucial for the reliable development of lithium-sulfur (Li–S) batteries.
On December 17, 2024, the team of Zeng Haibo and Li Gaoran from Nanjing University of Science and Technology published a research paper titled “Phosphorus-Tuned Nickel Boride Nanocatalyst with Enhanced p–p Interaction for Expediting Sulfur Electrochemistry in Lithium-Sulfur Batteries” in the journal Advanced Functional Materials, with team member Li Hongyang as the first author, and Hong Kong Polytechnic University’s Wang Huimin, Zeng Haibo, and Li Gaoran as co-corresponding authors.
This research proposes a phosphorus doping strategy to finely tune the electronic properties of nickel boride (P-NiB) to promote efficient sulfur electrocatalysis. By combining theoretical modeling with experimental validation, it was demonstrated that the electronegativity of P can reduce the 2p orbital occupancy of B, and the enhanced π-π orbital interaction facilitates the bonding of B─S. Meanwhile, P atoms and Ni atoms exhibit strong affinities for polysulfides through π-π and π-d interactions, forming an overall sulfur-philic catalyst that effectively limits polysulfides and reduces the conversion energy barrier. This enables the oxidation-reduction reaction of sulfur to be rapid and enduring. Based on P-NiB, the Li–S batteries outperform undoped NiB and bare carbon counterparts in cycling performance (1000 cycles) and rate performance (5C). Additionally, under high load and poor electrolyte conditions, a high specific capacity of up to 8.94 mAh cm−2 was achieved, demonstrating a pouch battery capable of providing a total capacity of 1.2 Ah.This research emphasizes an efficient anion regulation strategy for optimizing sulfur electrocatalysis based on metal borides, providing a promising approach for developing high-performance and practically feasible Li–S batteries.
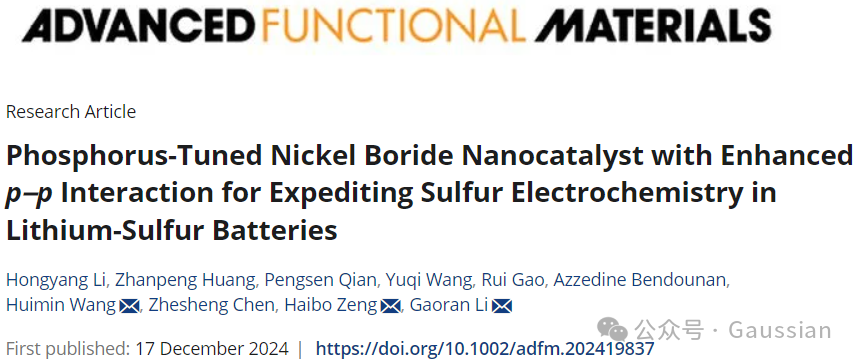
DOI:10.1002/adfm.202419837
The researchers proposed an anion regulation strategy, developing phosphorus-doped nickel boride as a superior catalyst for Li–S battery performance. Theoretical studies first predicted that due to the ionic nature between B and P, electrons would transfer from B to P. This redistribution of electrons helps reduce the occupancy of B‘s 2p orbitals, facilitating the acceptance of electrons from S, thus generating stronger B—S π-π interactions. Meanwhile, the 3p orbital of P and the 3p orbital of S exhibit π-π interactions, resulting in good sulfur affinity for the phosphorus dopant. Therefore, P-NiB demonstrates triple sulfur affinity, meaning that the three elements of Ni, B, and P collectively contribute to the fixation and catalysis of S. Guided by this prediction, an amorphous P-NiB was experimentally prepared through a simple solution method. As expected, a series of physicochemical and electrochemical characterizations confirmed that P-NiB exhibited excellent polysulfide adsorption capacity and catalytic activity. Sulfur electrodes equipped with P-NiB nanocatalysts demonstrated outstanding cycling and rate performance, and exhibited good performance under practical application conditions such as high sulfur loading, poor electrolyte, and pouch battery construction.
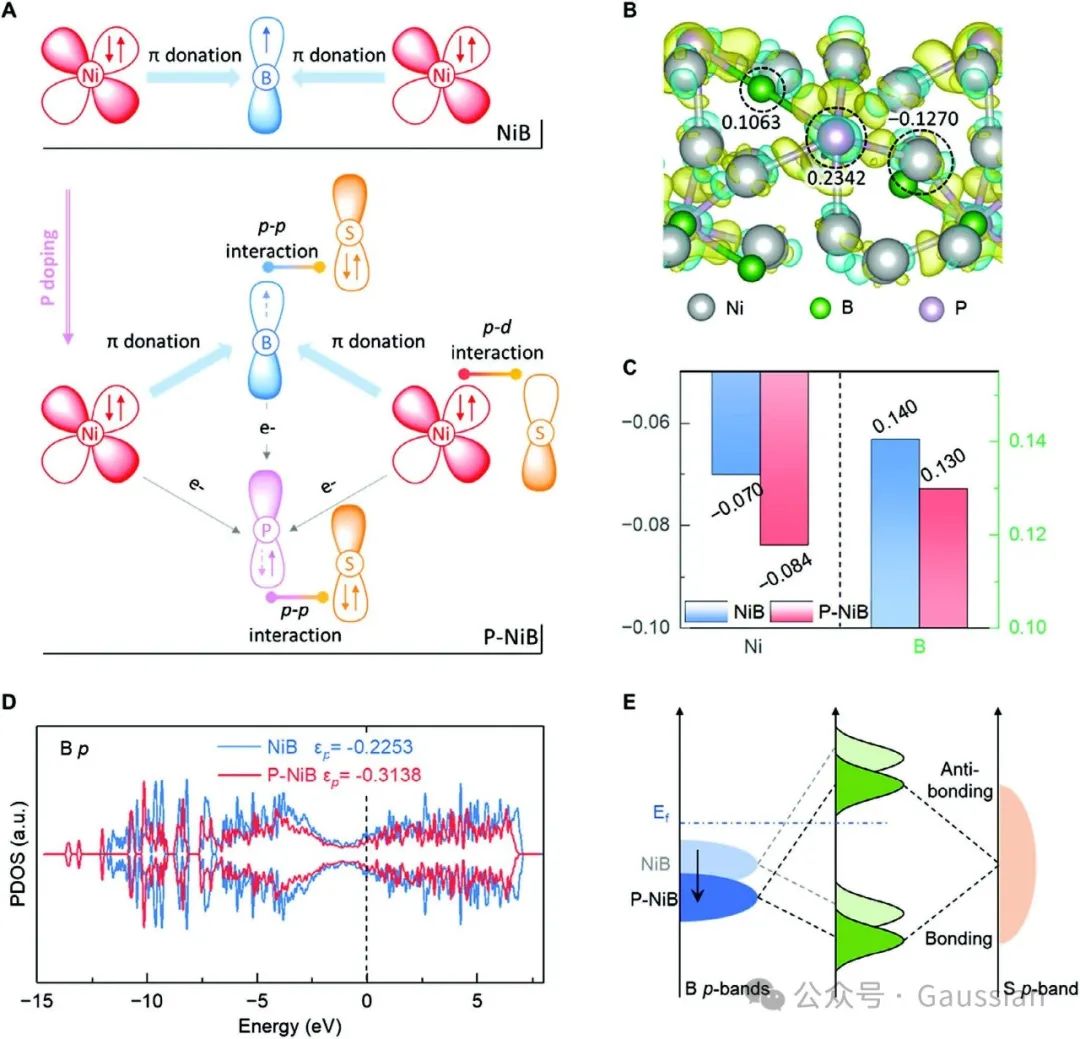
Figure 1. A) Schematic diagram of charge transfer and enhanced orbital interactions in P-NiB; B) Differential charge density of P-NiB and representative Ni, B, and P Barder charge;C) Average Barder charge of Ni and B in NiB and P-NiB; D) Density of states diagrams of NiB and P-NiB; E) Schematic diagram of orbital interactions with polysulfides.
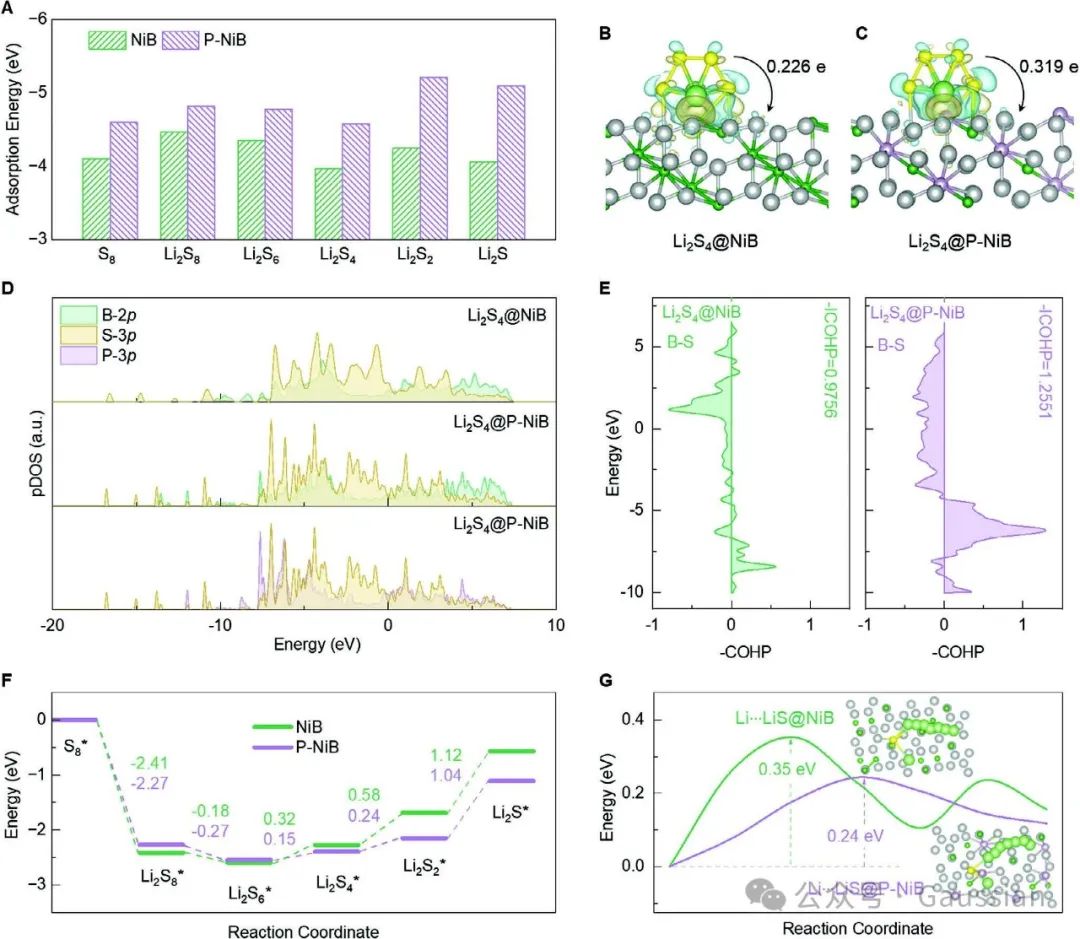
Figure 2. A) Adsorption energy of sulfur species on NiB and P-NiB; B) Geometrically stable configurations of Li₂S₄ adsorbed on the surface of NiB and P-NiB; C) B-2p, S-3p, and P-3p partial density of states diagrams of different Li₂S₄@ catalyst systems; D) Crystal orbital Hamiltonian occupancy diagrams of different Li₂S₄@ catalyst systems; E) Gibbs free energy of sulfur reduction reactions on different catalyst surfaces, and G) Gibbs free energy of Li₂S decomposition reactions on different catalyst surfaces.
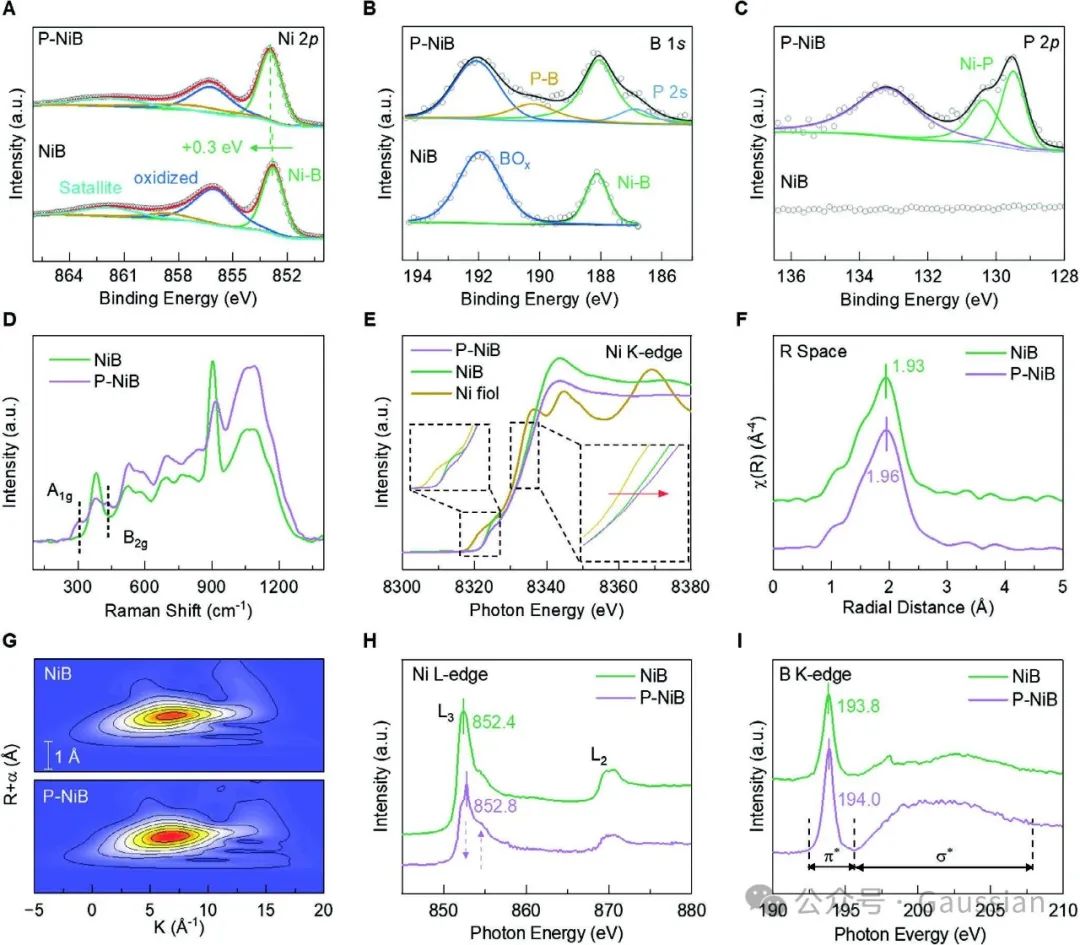
Figure 3. A) SEM images of P-NiB and C) NiB; D) TEM images of P-NiB and SAED images (insets); E) HRTEM images of NiB and P-NiB; F) EDS elemental distribution images of P-NiB.
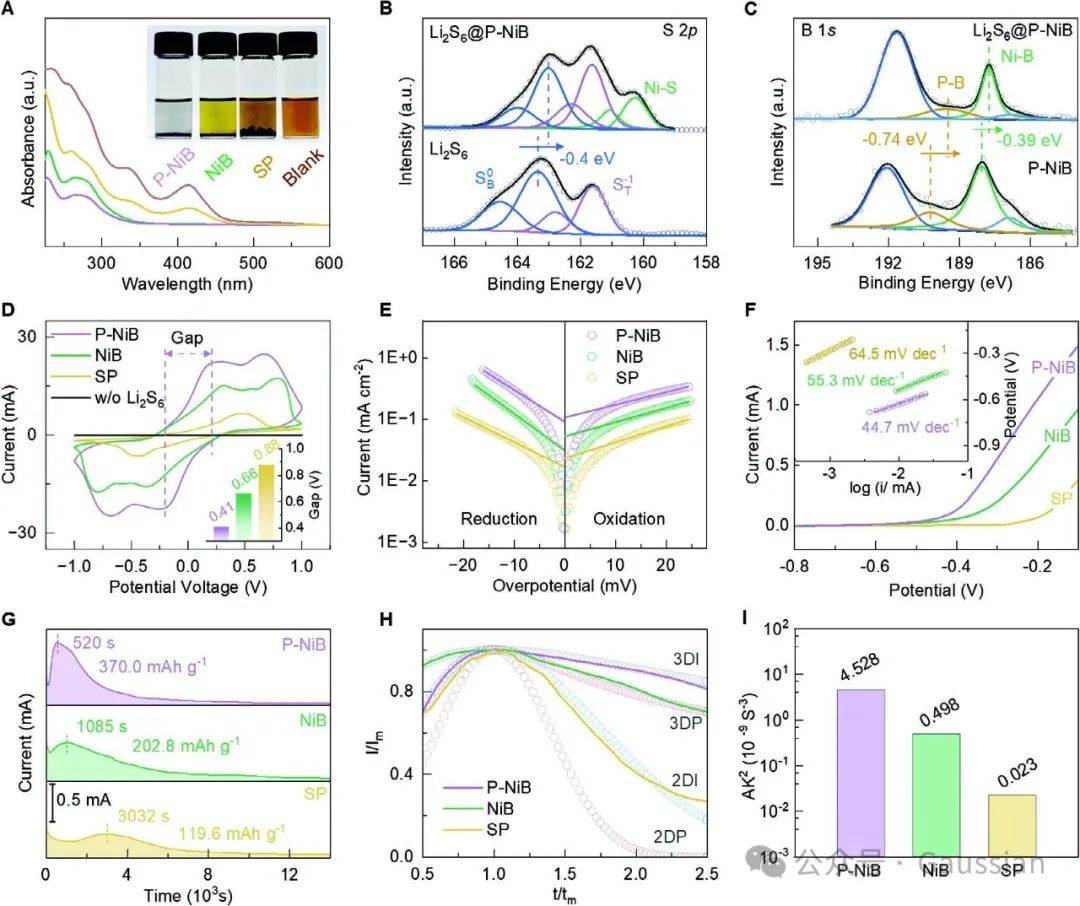
Figure 4. A) Ni-2p, B) B-1s, C) P-2p XPS spectra of NiB and P-NiB; D) Raman spectra; E) Ni K-edge EXAFS spectra; F) k³ weighted Fourier transform of Ni K-edge EXAFS spectra; G) Wavelet transform of Ni K-edge EXAFS spectra; H) Ni L-edge spectra; I) B K-edge spectra.
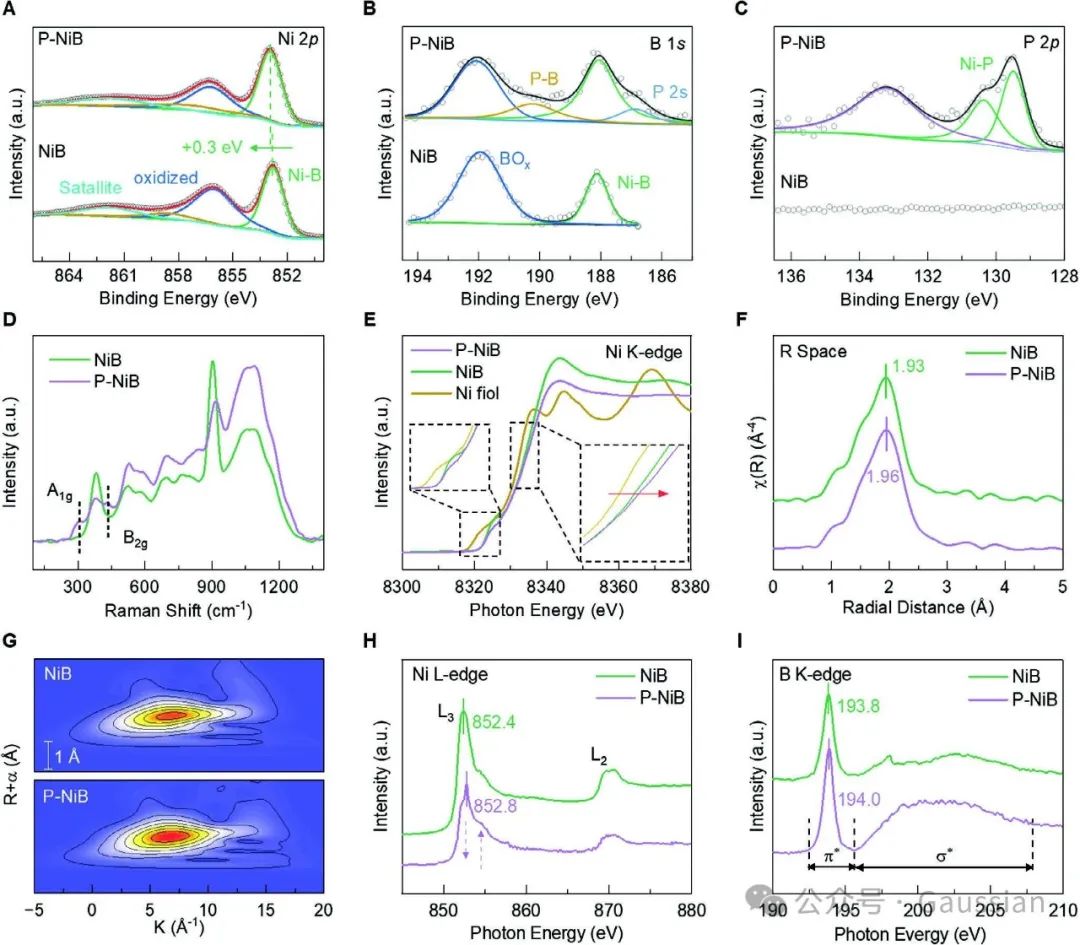
Figure 5. A) Adsorption of Li₂S₆ solution on different samples under UV-visible spectra and optical images (insets); B) S-2p and C) B-1s XPS spectra of P-NiB adsorbing polysulfides; D) CV curves of symmetric batteries based on different catalysts, and E) Tafel curves; F) LSV curves of Li₂S oxidation on different electrodes and the corresponding Tafel slopes (insets); G) Precipitation curves of Li₂S under constant potential, H) corresponding dimensionless transient conditions compared with two-dimensional and three-dimensional theoretical models.
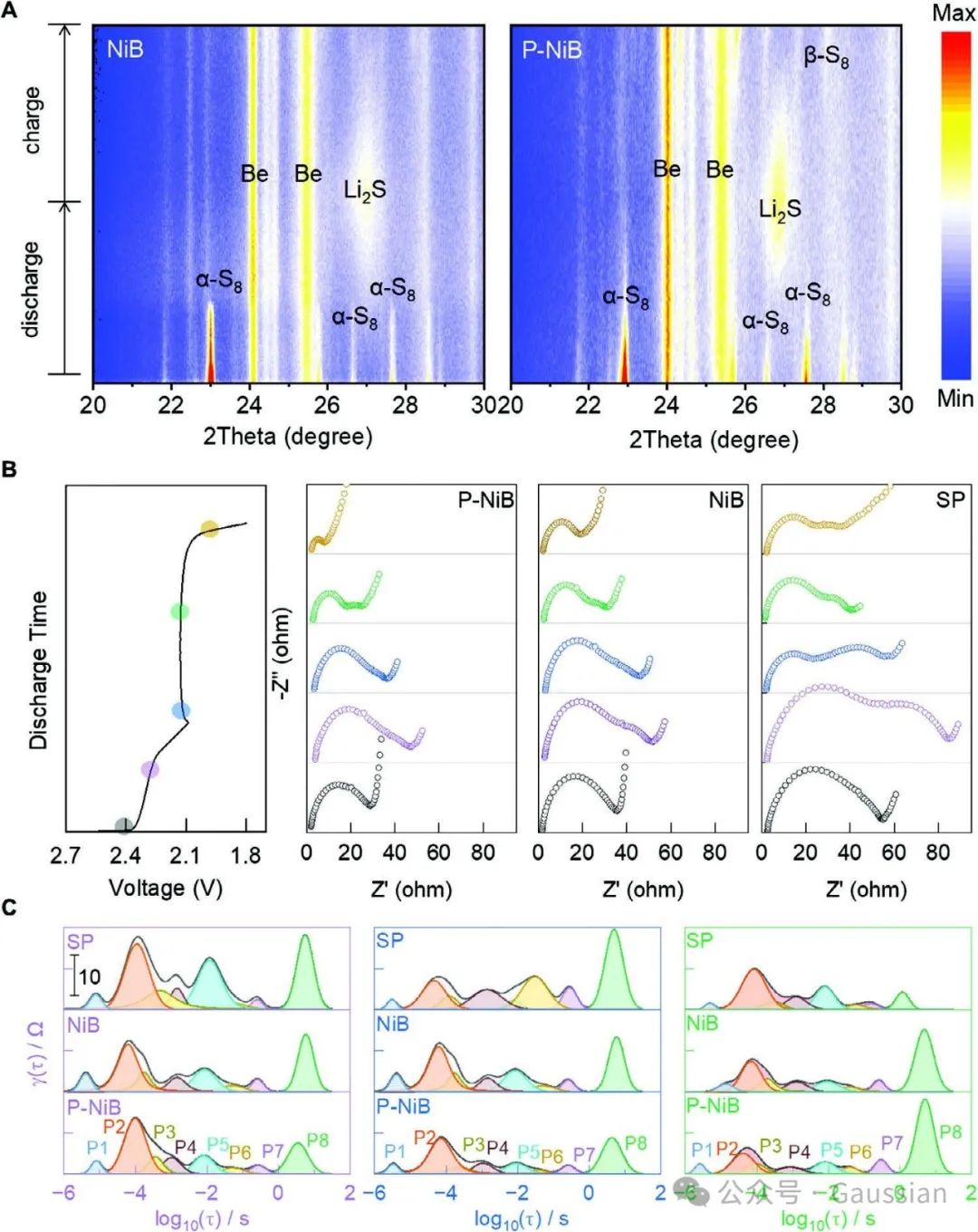
Figure 6. A) Nyquist plots of lithium-sulfur batteries based on SP, NiB, and P-NiB; B) their CV curves; C) Reduction of activation energy for sulfur conversion by NiB and P-NiB; D) Charge-discharge curves of different batteries; E) Cycling performance of different batteries; F) Charge-discharge curves of P-NiB batteries at different current rates; G) Rate performance of different batteries; H) Long-term cycling performance of different batteries.
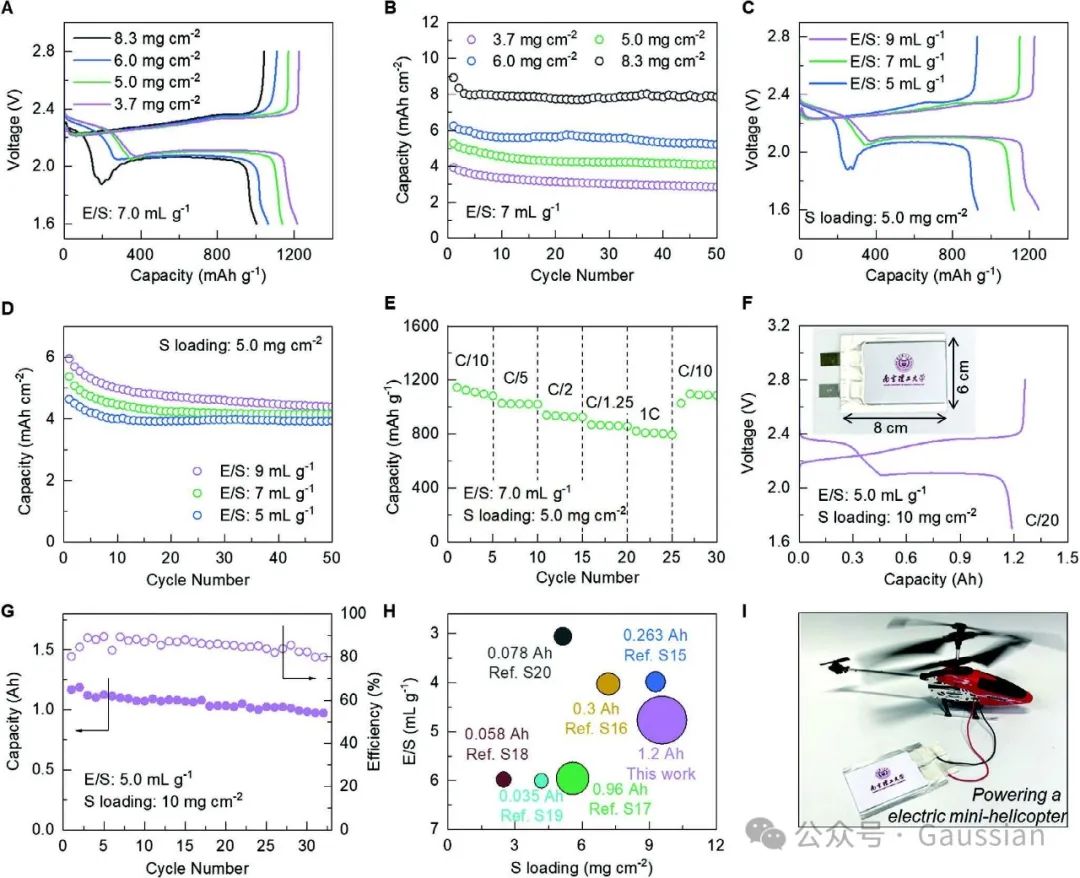
Figure 7. A) In situ XRD patterns of NiB and P-NiB batteries; B) Nyquist plots of SP, NiB, and P-NiB batteries under different discharge states, and C) corresponding relaxation time distribution plots.
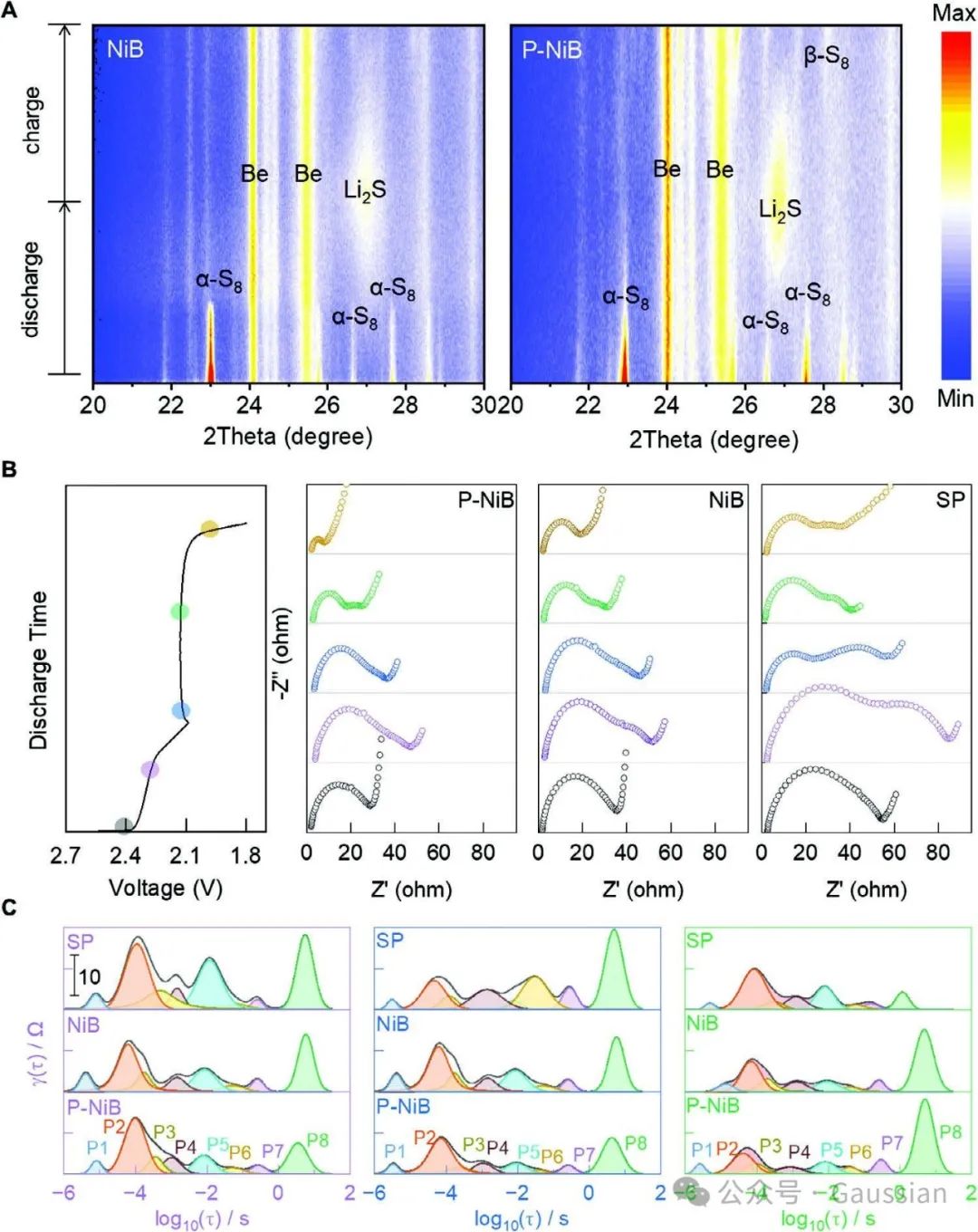
Figure 8. A) Voltage curves of P-NiB batteries under different sulfur loadings and B) cycling performance; C) Voltage curves and D) cycling performance of P-NiB batteries under different electrolyte/sulfur (E/S) ratios; E) Rate performance of high loading P-NiB batteries; F) Voltage curves of lithium-sulfur pouch batteries based on P-NiB and G) capacity; H) Key parameters of P-NiB pouch batteries compared with recent literature; I) Appearance photo of a small electric helicopter powered by P-NiB pouch batteries.
In summary, this research developed an anion regulation strategy to adjust the electronic structure of nickel boride and enhance its catalytic activity to achieve excellent sulfur electrochemical performance. The introduction of P effectively redistributes electrons, enhancing the π-π orbital interactions with polysulfides. Meanwhile, P and Ni participate in binding to sulfur species through π-π and π-d interactions, collectively forming a unique catalyst with global sulfur-philicity for sulfur reactions. Comprehensive physicochemical and electrochemical analyses confirmed the electron regulation effect in the synthesized P-NiB catalyst, as well as its enhanced polysulfide adsorption capacity and catalytic activity. The resulting rapid and enduring sulfur electrochemical performance enables Li–S batteries based on P-NiB to possess excellent cycling performance (over 1000 cycles), outstanding rate performance of up to 5C, and robust performance even under harsh conditions (including high sulfur loading of 8.3 mg cm−2, or low electrolyte/sulfur ratios of 5.0 mL g−1) as well as a pouch battery with a capacity of 1.2 Ah, providing good energy density for powering electronic devices.This research provides an effective anion regulation strategy for designing advanced sulfur-philic sulfur electrocatalysts, with significant application prospects in the development of high-performance and practically feasible Li–S batteries.
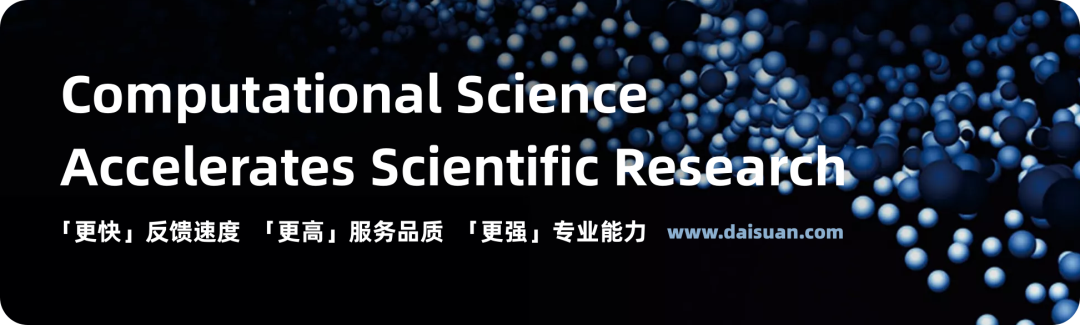
■ Density functional theory (DFT) calculations: charge density, density of states (DOS), energy bands, Fermi level, work function, ELF; dielectric constant, elastic modulus, phonon spectrum; Gibbs free energy, adsorption energy, doping energy, defect formation energy; HER, OER, ORR, NRR, CO2RR; reaction pathways, reaction mechanisms, migration energy barriers, etc.
■ Quantum chemistry (QC) calculations: electrostatic potential, dipole moment, occupancy, orbital characteristics, spin density, Fukui function; excited states, transition dipole moments; hydrogen bonds, π-π stacking, hydrophobic forces; transition states, reaction energy barriers, reaction mechanisms; infrared, Raman, fluorescence, phosphorescence, NMR, circular dichroism, etc.
■ Molecular dynamics (MD) simulations: analysis of weak interactions in biological systems, receptor-ligand assembly processes, binding free energy; prediction of polymer conformations in material systems, properties of material-solution interfaces, coarse-grained simulations; trajectory analysis (RMSD/RMSF), radial distribution function (RDF), diffusion, number of hydrogen bonds; molecular docking; homology modeling; virtual screening, quantitative structure-activity relationships (QSAR).
■ Finite element method (FEM) simulations: structural simulations (contact analysis, nonlinear analysis, vibration/fatigue/heat transfer/crack/collision analysis); electromagnetic simulations (electric field, magnetic field, electromagnetic coupling, magnetothermal coupling, RF microwave); fluid simulations (multiphase fluid, component transport, fluid dynamics, phase change); optical/acoustic simulation related.