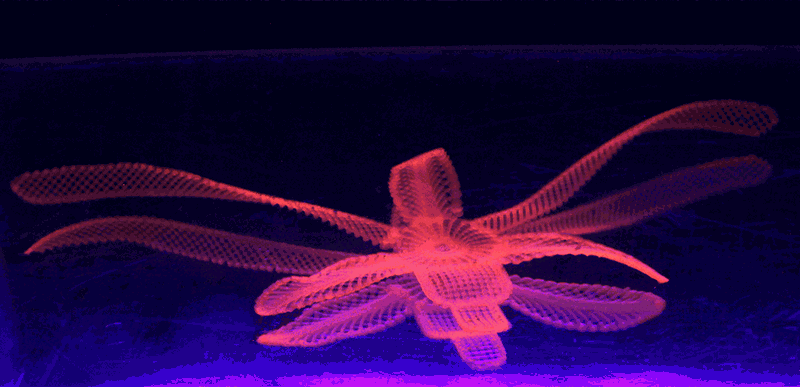
Figure 1 4D printing model source: https://3dprintingindustry.com/news/65379-65379/
In 2013, American scholar Skylark Tibbits first proposed the concept of 4D printing in a TED talk titled The emergence of “4D printing”: complex 3D printed structures can change their configurations over time. The first paper on 3D printing was published in 2013 in the journal Applied Physical Letters, reporting on a 3D printed structure with temperature-controlled deformation, made using shape memory PAC (polyacrylate) through SLA technology [2].
In recent years, research on 4D printing has attracted close attention in the fields of smart materials and additive manufacturing, and it has developed rapidly. The concept has also been continuously enriched as research deepens. Generally speaking, 4D printing can be simply understood as “3D + time”; broadly speaking, in addition to shape changes, the complex shapes produced by 3D printing that respond to external stimuli to produce performance and functional changes can also be referred to as 4D printing. The external stimuli mainly include heat, magnetism, light, humidity, pH, etc. The core of 4D printing technology lies in programmable and designable structures that can change over time under specific conditions, and its realization relies on two important foundations: mature additive manufacturing technology (i.e., the 3D printing we are familiar with) and smart materials that meet the functional requirements of 4D printing (often materials with shape memory effects).
Additive manufacturing technology (hereinafter referred to as 3D printing) is no stranger to us. It connects discrete raw materials into a three-dimensional whole through point-by-point scanning and layer-by-layer stacking, combined with computer-aided structural design, enabling the direct manufacture of various complex geometric structures while maximizing material savings and reducing subsequent processing. From the perspective of forming principles, the commonly used 3D printing technologies include photopolymerization for polymer materials (Stereolithography, SLA), fused deposition modeling (Fuse Deposition Modelling, FDM), direct ink writing (Direct Ink Writing, DIW), and selective laser melting/sintering for metal materials (Selective Laser Melting/Sintering, SLM/SLS), among others. After decades of development, it has now matured to achieve the forming and manufacturing of complex fine structures in metals, polymers, and ceramics, and has been applied in various fields such as aerospace, biomedical, mechanical electronics, industrial design, and cultural education [3]. However, as the demand in application fields continues to expand, the functional designability of static structures or components produced by traditional 3D printing has shown certain limitations. For example, self-folding smart devices from micro to macro scales are considered to have good application prospects in fields like biomedicine and aerospace, yet are difficult to realize through traditional materials 3D printing.
Naturally, scientists have thought of combining smart materials with shape memory effects, self-healing materials, or “metamaterials” with special topological structures with 3D printing technology to maximize the functional and structural design advantages of materials, thereby giving traditional 3D printing technology the wings of designability in the time dimension—it can be said that the emergence of 4D printing enriches the potential and vitality of 3D printing technology. [4, 5]
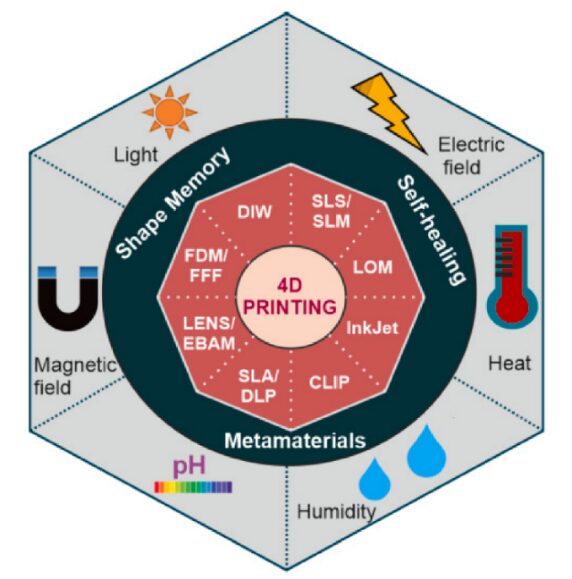
Figure 2 The relationship between 4D printing technology, materials, and external action fields [5]
Thermal Responsive Material 4D Printing
4D printing of thermal responsive materials is relatively mature in terms of materials and processes. Thermal responsive materials are based on material phase transitions or glass transition, corresponding to phase transition temperature Tm or glass transition temperature Tg. In 4D printing, materials are formed above the phase transition temperature or glass transition temperature through 3D printing, and then external force deformation is applied below the phase transition temperature to perform secondary configuration, at which point the morphology is a temporary shape. The temporary shape can be maintained below the phase transition temperature. When the temperature returns above the phase transition temperature, the shape restores to the printed shape. Currently, a wide range of thermal responsive materials can be used for 3D printing, including shape memory materials, hydrogels, liquid crystal elastomers, etc., with the process of shape memory materials being relatively mature. One classic example is the deformation of the 3D printed Eiffel Tower model at specific temperatures [6].
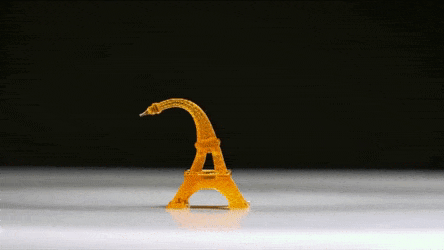
Figure 2 Deformed Eiffel Tower [6]
Water Responsive Material 4D Printing
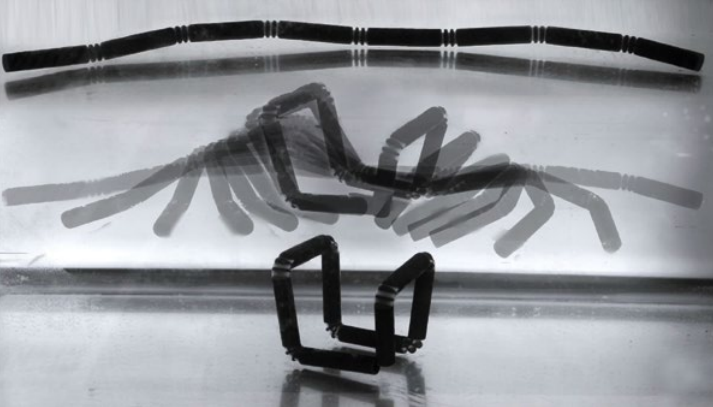
Figure 3 Bending deformation of materials with different expansion rates in water [7]
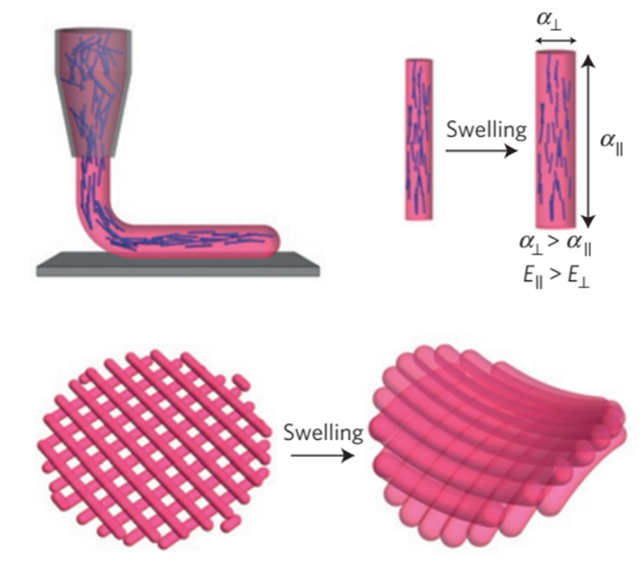
Figure 4 Different expansion rates caused by directional arrangement of nanofibers [1]
Magnetic Responsive 4D Printing
Magnetic responsive 4D printing is achieved by doping magnetic particles into the printing materials, which are driven by forces in a magnetic field to deform the overall structure. To achieve significant deformation of the 3D printed structure in a magnetic field, soft materials are generally chosen, such as graphene or PDMS. However, under a magnetic field, the overall structure can be quite “powerful”. For example, a PDMS robotic hand with doped magnetic particles can lift objects exceeding 1000 times its own weight [1]. This is due to the interaction forces between the magnetic particles, which increase the stiffness of the overall structure. In 2018, the academic journal Science Advances reported on mechanical metamaterials for 4D printing. Researchers first used a 3D printer to print a hollow polymer shell, then filled it with magnetorheological fluid. The magnetorheological fluid becomes magnetized in a magnetic field, producing interaction forces that cause the overall structure to exhibit different stiffness under different magnetic fields, achieving mechanical performance responsive to external stimuli [8].
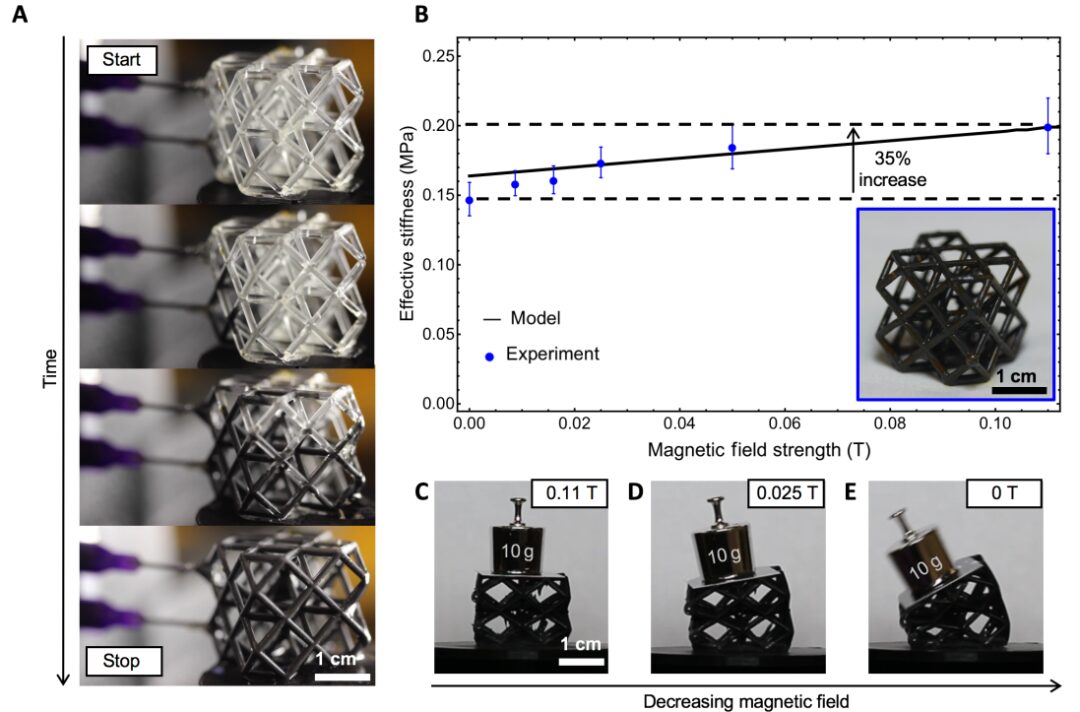
Figure 5 Mechanical metamaterials responsive to magnetic fields [8]
Ceramic Material 4D Printing
The vast majority of 4D printing technologies are based on polymer materials, with few reports on ceramic 4D printing. This is because the 3D technology for ceramics uses ceramic slurries or precursors, making it difficult to achieve significant deformation after forming. Professor Lü Jian from City University of Hong Kong led a team to take a different approach, achieving ceramic 4D printing for the first time. This achievement was published in the renowned academic journal Science Advances [9].
Unlike traditional ceramic slurries or precursors, this team uniformly mixed nanoscale ceramic particles with a polymer elastomer (dimethylsiloxane) to create a ceramic composite elastomer that is highly elastic and can recover after being stretched by 200%. Researchers printed pre-set patterns using direct ink writing (DIW). The ceramic 4D printing consists of two parts: the main structure and the substrate. Researchers apply pre-stress to the substrate and connect it with the main structure; after releasing the substrate stress, the main structure deforms accordingly. This special structure, which undergoes deformation due to pre-stress, must undergo high-temperature sintering to obtain the final ceramic product. The ceramic 4D printing technology has unique advantages in obtaining complex curved surfaces, and combined with the inherent properties of ceramics such as electromagnetic shielding and corrosion resistance, ceramic 4D printing is expected to shine in the 5G era.
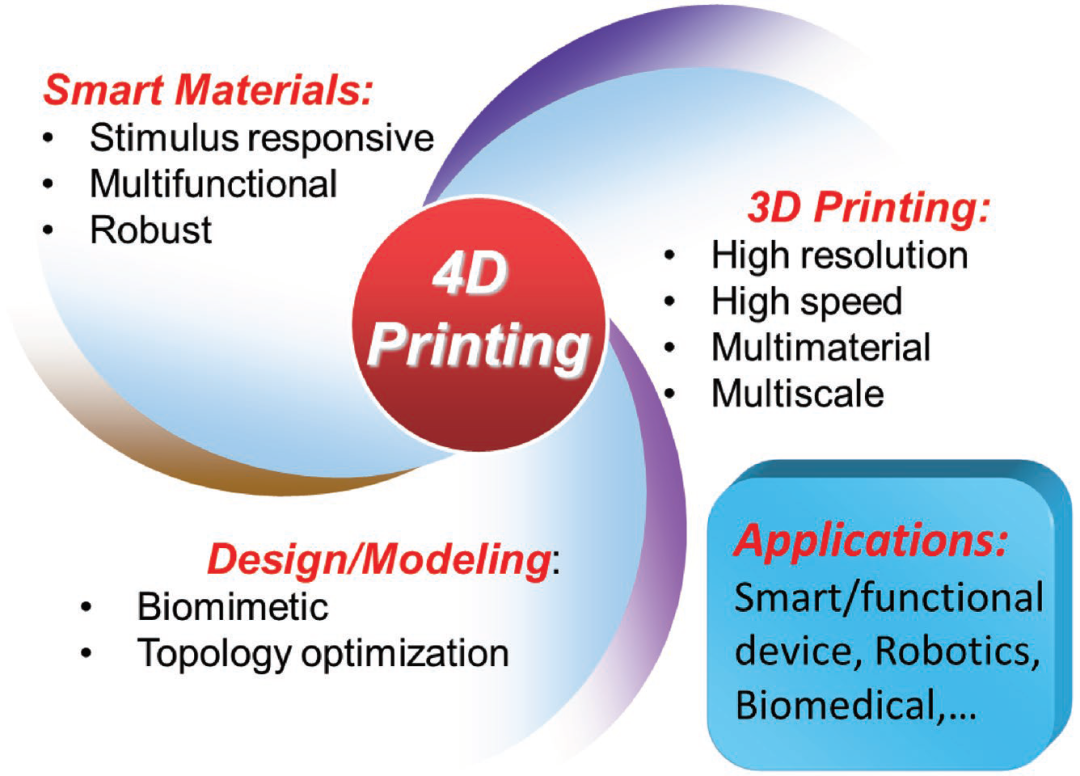
Figure 6 Future development demands and applications of 4D printing technology [4]
Currently, 4D printing technology, as a young research field, has most reported studies still in the exploratory stage, with a certain distance from practical production applications. In the future, the further development of 4D printing will rely on technological advancements and in-depth research across multiple disciplines: first, the rapid preparation of functional materials or devices with complex structures across scales still relies on the development of high-precision, high-speed, multi-material 3D printing technology; second, new multifunctional ink materials suitable for 4D printing need further development; third, there is a need to establish systematic theoretical models and design methods to accurately predict and optimize the topological shape changes of 4D printed components. It is undeniable that this new technology has already demonstrated its tremendous application potential in various fields, such as smart devices, intelligent packaging, self-folding materials, metamaterials, and biomedical engineering [4, 5]. The following are two examples for a brief introduction:
(1) Flexible Robots
Flexible robots are currently one of the core areas of focus for 4D printing applications. The programmable characteristics of shape memory materials mean that we can print materials into devices with driving capabilities. For example, in extreme environments such as high altitudes, outer space, floods, and snow, as long as appropriate structural designs and stimulus-response methods are identified, it is possible to design such materials to mimic biological responses to environmental changes, creating programmable smart flexible robots with various functions [5].
(2) Biomedical Applications
The additive manufacturing of biomedical materials has developed rapidly in recent years, so materials related to biomedicine such as shape memory alloys, polymers, and hydrogels have shown great potential in customization and personalized manufacturing, making 4D bioprinting particularly promising in biomedical applications. For example, 4D printing can be used to create customized sutures, vascular repair devices, and to develop dynamic biomedical devices for drug delivery, electronic skin, and biomimetic robots, mimicking applications in biological systems (such as complex artificial muscles) [4,5].
References
[1]Gladman A S, Matsumoto E A, Nuzzo R G, et al. Biomimetic 4D printing[J]. Nat Mater, 2016, 15(4): 413-8.
[2]Ge Q, Qi H J and Dunn M L. Active materials by four-dimension printing[J]. Applied Physics Letters, 2013, 103(13): 131901.
[3]Ngo T D, Kashani A, Imbalzano G, et al. Additive manufacturing (3D printing): A review of materials, methods, applications and challenges[J]. Composites Part B: Engineering, 2018, 143: 172-196.
[4]Kuang X, Roach D J, Wu J, et al. Advances in 4D Printing: Materials and Applications[J]. Advanced Functional Materials, 2019, 29(2): 1805290.
[5]Ryan K R, Down M P and Banks C E. Future of additive manufacturing: Overview of 4D and 3D printed smart and advanced materials and their applications[J]. Chemical Engineering Journal, 2021, 403.
[6]Ge Q, Sakhaei A H, Lee H, et al. Multimaterial 4D printing with tailorable shape memory polymers[J]. Scientific reports, 2016, 6(1): 1-11.
[7]Tibbits S. 4D printing: multi‐material shape change[J]. Architectural Design, 2014, 84(1): 116-121.
[8]Jackson J A, Messner M C, Dudukovic N A, et al. Field responsive mechanical metamaterials[J]. Science advances, 2018, 4(12): eaau6419.
[9]Liu G, Zhao Y, Wu G, et al. Origami and 4D printing of elastomer-derived ceramic structures[J]. Science advances, 2018, 4(8): eaat0641.
Author | Zhao Ruoshi, a 2019 PhD candidate at Tsinghua University, specializing in structural ceramics and ceramic 3D printing. Zhang Wenqiang, a doctoral student at the Department of Mechanical Engineering, City University of Hong Kong, specializing in 3D printing of mechanical metamaterials.
Typesetting | Zhao Ruoshi, Yu Yihang
Editing | Kou Fangcheng, Wei Kun
Review | Zhang Keren
This article is reproduced with permission from Tanzhen Technology Review (ID: TWtechreview). For secondary reproduction, please contact the original author.
Welcome to share in your social circles.